Charge Carrier Mobility in organic semiconductors
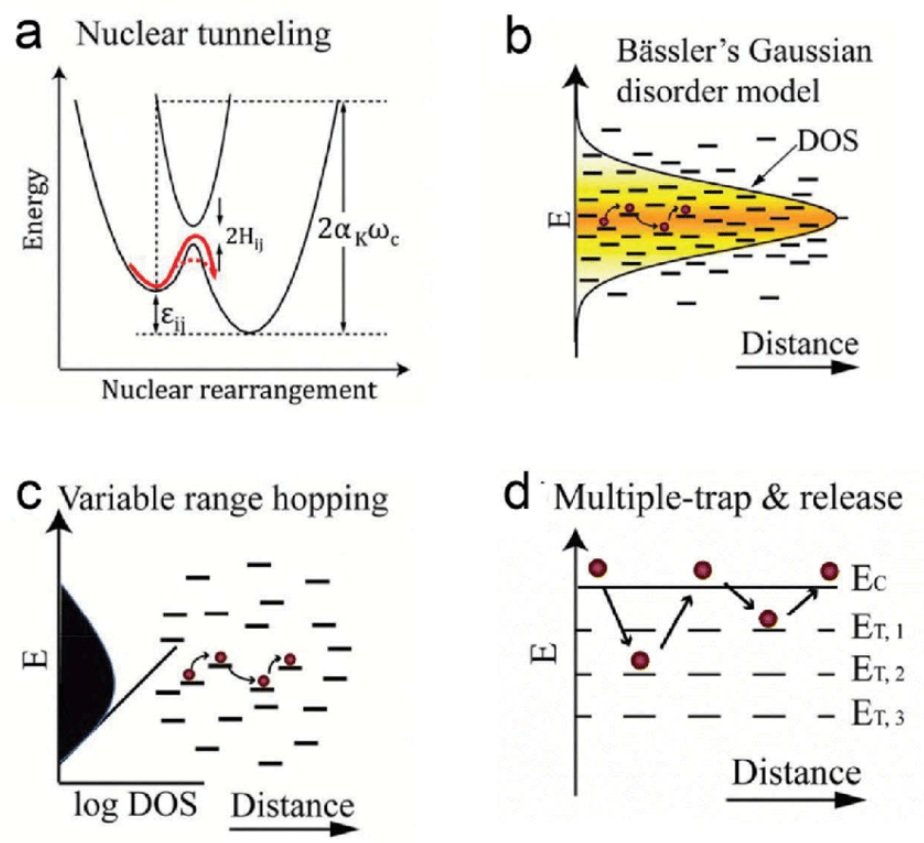
Charge carrier mobility is one of the most important parameters in organic and perovskite semiconductors used in solar cells and light-emitting devices.
This blog post first summarizes the different techniques that are used to extract carrier mobility. Then, it will focus on how to extract it from the IV characteristics of single-carrier devices (SCDs). Device simulations are used as synthetic data to analyze the requirements and limitations of the analytical Mott-Gurney law.
Introduction
Various experimental techniques are used to extract the charge carrier mobility. Interesting techniques are time-resolved terahertz spectroscopy, time-resolved microwave conductance, CELIV, and space-charge-limited current (SCLC). [1] Even if we limit ourselves to the electrical measurements available in our instrument PAIOS, there are different methods to choose from. All these methods have their specific requirements, advantages, and disadvantages.
An overview of techniques is given in Table 1.
Techniques to Determine the Charge Carrier Mobility in Semiconductors. | |||
---|---|---|---|
METHOD | MEASUREMENT | DEVICE | REQUIREMENTS |
Space Charge Limited Current (SCLC) | Current-Voltage (steady-states) | Unipolar (Single-carrier Device) | Ohmic Contacts |
Dark-Injection Transient (DIT) [1] [2] | Voltage Pulse (Transient) | Unipolar (Single-carrier Device) | Ohmic Contacts |
Charge Extraction By Linar Voltage (CELIV) [3] | Voltage Ramp (Transient), light pulse | Bipolar (diode) Metal-Insulator-Semiconductor (MIS) | < 1 non-injecting blocking contact |
Time of Flight (ToF) [4] | Light and Voltage Pulses (Transient) | Unipolar Single-Carrier Device or Bipolar | Ohmic contacts (energetically aligned) |
Transient Electroluminescence (TEL) [5] | Voltage Pulse (Transient) | OLED | Energetically disaligned contacts |
Negative Differential Susceptance [6] [7] | Impedance Spectroscopy | Unipolar (Single-carrier Device) | Ohmic Contact |
Most experiments are performed on either hole- or electron-only single-carrier devices (SCDs). These devices consist of a single semiconducting layer sandwiched between two electrodes – optionally with additional charge injection and/or -transport layers.
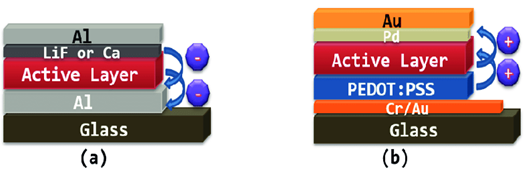
Figure 1: representive device structure of a single-carrier device (SCD). a) electron-only devie, b) hole-only device.
When extracting the charge carrier mobility from any experimental technique, it is important to understand “which carrier mobility” they can give. It is also important to ensure that the structure and fabrication processes of the device used to determine the mobility are comparable to those of the real sample. Different techniques probe different timescales (after photoexcitation) and may be affected by thermalisation effects. [8] Moreover, if the interest is extracting the mobility of the active layer of a solar cell, the values extracted by measuring a Field-Effect Transistor (FET) should not be used (because the FET gives the in-plane mobility). Also, it would be necessary to exclude the Time-of-Flight (ToF) because this requires devices with a semiconducting layer thickness > 1 µm (rarely achievable from solution processing techniques typically used for thin-film devices).
Using the Mott-Gurney model (MG) to analyse the IV characteristics of SCDs is probably the most popular technique to extract the mobility of an organic semiconductor sandwiched between two electrodes. Beyond The standard MG model has also been extended by the inclusion of several contributions: the influence of distributed trap states, the effects of the electric field on the trap depth, and the effect of non-ohmic metal/insulator interfaces. [9][10]
Generally, these models are complex mathematical formulations that do not provide a unique description of the material under analysis. However, when only discrete trap levels influence the space charge current, simple analytical equations are available, and several parameters regarding the charge carrier mobility, the density of thermal free carriers and the density/depth of charge traps can be obtained by the analysis of a single IV characteristic. The advantage of the Mott-Gurney model is that it gives a straightforward equation with few variables. However, this must be used very carefully because the derivation of this equation is subjected to several preliminary assumptions in the model.
This blog aims to clarify to what extent the Mott-Gurney model can be used. We will review the MG model's limitations using device simulations and make specific recommendations for experimentalists.
Background of Analytical Models for IV Data
The Space charge limited current theory describes the steady state response of an organic material (or, in general, of an insulator) to an applied bias. Charge injection in a conventional semiconductor occurs without the accumulation of charge because of the high mobility of the charge carriers. On the other hand, an organic semiconductor is usually quite an electrically insulating material with a low density of thermally generated free carriers n0. As soon as the density of the injected charges is higher than n0, the material becomes charged with a stationary space charge that, depending on the properties of the materials, can be free or trapped in localized states. This space charge strongly influences the electric field distribution that, in this case, is not constant through the bulk of the material.
Following the descriptions by Röhr et al. [12], the IV curve of an SCD can be divided into three different regimes. However, as the authors show, not all three regimes can be seen in many cases.
In the low voltage regime, the current depends linearly on voltage. In the case of trap and doping-free semiconductors and non-limited charge carrier injection, the mobility can be inferred from this region using (Equation 1): [13]
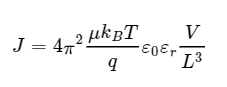
Here, q is the elementary charge, µ is the mobility, kBT is the thermal energy, ε0 and εr are the vacuum and relative permittivity, respectively, and L is the layer thickness. If the semiconductor is (unintentionally) doped, the current can also appear linear with voltage due to Ohmic conduction. In this case, the analytical formula reads (Equation 2): [14]
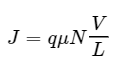
Where N is the carrier concentration. The different thickness scaling should be noted here. This different scaling with thickness can be used to assess whether a material is unintentionally doped (1/L) or governed by diffusion (1/L3), given that multiple layer thicknesses are available.[15] However, the required doping concentration to evaluate the charge carrier density correctly needs to be rather high (> 10^18 cm-3). [14]
In the intermediate voltage regime, when diffusion of charge carriers becomes negligible, the well-known Mott-Gurney (MG) equation describes the current-voltage relation (Equation 3): [16]
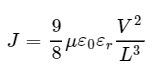
The analytical model is only valid if the following conditions are met [12]: (i) negligible intrinsic charge-carrier concentration (no doping), (ii) Ohmic contacts (no barriers), (iii) no traps or energetically disordered states, (iv) current is only composed of drift.
In the high voltage regime, the current can become again linear with voltage and be described by (Equation 4): [12]
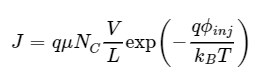
Where Nc is the density of charge carriers, and qϕinj is the energetic barrier at the electrode.
Testing the limit of the Mott-Gurney model with Setfos
There are quite a few publications in which the limitations of the previous analytical formulas have been analysed. Often, this is done by generating synthetic data using drift-diffusion device models and comparing the extracted mobility with the input value. The above-mentioned paper by Röhr et al., presents an extensive overview on the validity of the MG equation for a range of device thicknesses in the case of trap states, non-Ohmic contacts and a combination thereof. In the case of no injection or extraction barrier, the slope of the JV curve only approaches 2 at high voltages (> 20 V) and eventually decreases even for devices < 100 nm at higher voltage. For existing barriers, the slopes even stay significantly below 2. The SCLC analysis still leads to reasonable mobility values, especially for thick devices (> 100 nm).
We performed drift-diffusion simulations with the software Setfos to analyse the limitations of the analytical MG law in special cases like the presence of injection barriers or electrical doping. Figure 2 shows the workflow of the performed consistency check. A model of the SCD is set up in Setfos to generate synthetic JV curves. We then fit the MG model to the simulated JV curve and compare the extracted mobility with the one we used in Setfos. This procedure allows us to assess the validity (range) of analytical models such as the MG law.
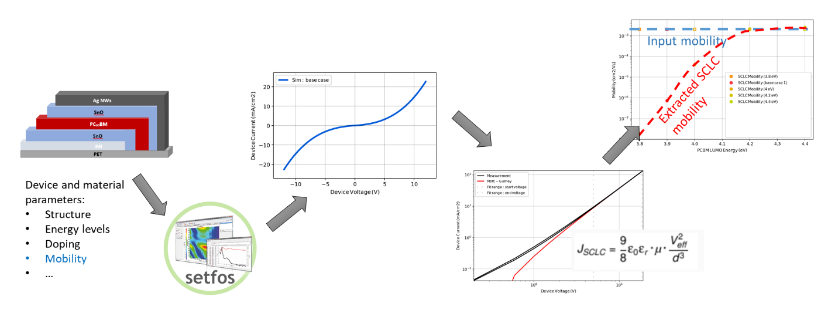
Figure 2. Sketch of a consistency check. A device model is setup in Setfos to generate synthetic JV curves. Applying the analytical model to this data and comparing the extracted parameter with the input value allows to assess the reliability of the analytical model.
The input parameters for the different simulations are shown in Table 2. The “base case” represents a very simple device structure without an energetic barrier. For all cases, the simulations are performed for three devices with thicknesses of 60, 120, and 240 nm. For the shunted device, we use a parallel resistor that is either fixed or varied with layer thickness.
Parameter |
Base (a) |
Barrier (b) |
Low mobility (c) |
Doping (d) |
Shunt (e) |
Field-enhanced mobility (f) |
Barrier 1 [eV] |
0 |
0.3 |
|
|
|
|
Barrier 2 [eV] |
0 |
0.1 |
|
|
|
|
Relative permittivity |
4 |
|
|
|
|
|
(Zero-field) Mobility [cm2/Vs] |
2e-3 |
|
2e-4 |
|
|
|
Field-enhancement coefficient [(m/V)1/2] |
- |
|
|
|
|
5e-4 |
Doping [cm-3] |
- |
|
|
1e17 |
|
|
Parallel Resistance [Ω] |
1e9 |
|
|
|
1(*d[nm]/60) |
|
Table 2. Input parameter used for the simulation of synthetic SCD JV data. For simplicity, only the changes with respect to the base case are shown.
JV Characteristics - Slope Analysis
Figure 3 shows the simulated JV curves and the analysed slopes (dlogJ/dlogV) as a function of voltage.
The theoretically expected slope of 2 is not reached in most cases but approaches the value for large voltages. In the case of an injection barrier of 0.3 eV (Figure 3b), the slope saturates at a value of 1, in line with findings by Röhr et al.[12] A slope considerably below 1 can occur in (thick) doped or (thin) shunted devices as well as for an injection barrier of ~0.3 eV. The latter can potentially be identified by the slope vs. voltage behaviour.
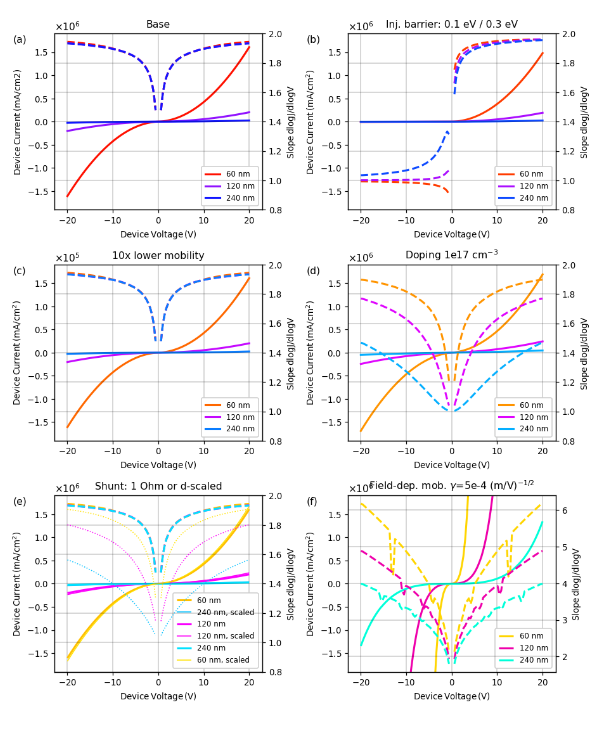
Fig. 3. Simulated JV (full line) and slope (dlogJ/dlogV) vs. voltage (dashed line) using six sets of parameters (see Table 2).
The doped devices and the thickness-scaled shunted devices show similar slopes, which are (well) below the theoretically expected value of 2. Therefore, the slope and the change in layer thickness are not a good indication to distinguish these two effects in the experiment. We also conducted further tests using simulations to analyse whether the doped devices could somehow be separated from a purely shunted device considering also other measurements. For example, whether the extracted geometrical capacitance and parallel resistance from electrical impedance spectroscopy would show significantly different trends for doped vs. shunted devices was analysed. However, both types of devices simply show roughly the same values.
Thickness scaling
As suggested in the literature [15], multiplying the current with L^-1 or L^-3 should shed light on the applicability of the MG law in the intermediate to high voltage range. This would allow us to distinguish between equations (1) and (2) for the low voltage regime.
Figure 4 shows the expected L^-3 scaling for the simulated cases (a) - (c).
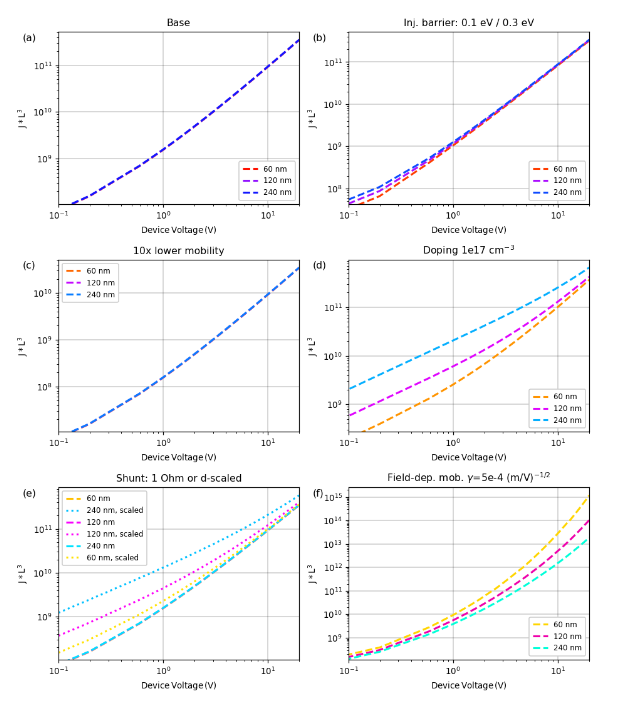
Figure 4. JL^3 scaling of the simulated JV curves.
The cases with slopes clearly below 2 (injection barrier 0.3 eV, doping, and scaled shunt) are further interesting to analyse. A barrier of 0.3 eV leads to a linear scaling with thickness (Figure 5a below). Surprisingly, the doping does not lead to a purely Ohmic conduction. The doping level of 1e17 cm-3 (1:10’000) is probably too low, which is also in line with previous publications. [5]
It is worth doing such scaling plots, as doping and the 0.3 eV barrier show a different JL behaviour.
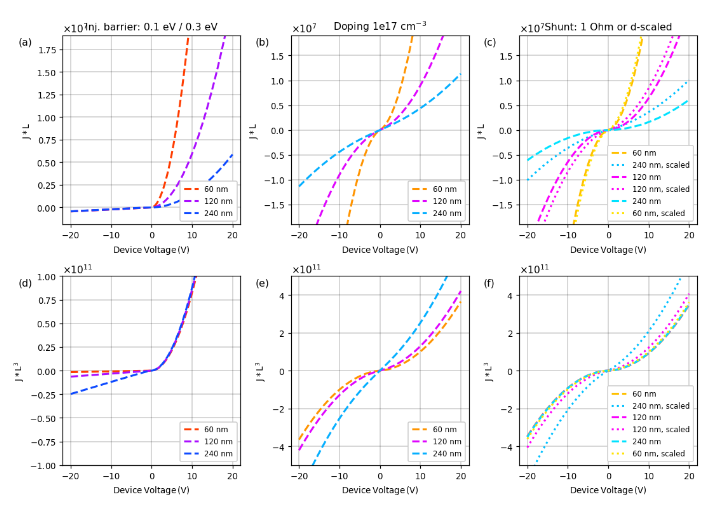
Figure 5. L and L^3 scaling of the cases barrier, doping and shunt.
Extraction of the carrier mobility with the Mott-Gurney law
To evaluate the validity of the mobility extraction with the MG model, we fitted equation (3) to the simulated JV curves (see Figure 3, using cases shown in Table 2) between 10 – 20 V. To quantify the error between the input mobility and the one extracted with the MG model, we define the relative error as (Equation 5):
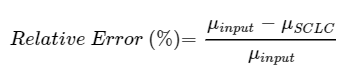
Figure 6 shows the value for all the different cases. For cases (a) – (d) the relative error is below 3%. Especially for situation (d), this is remarkable, as the analysed slope is – depending on the thickness – between 1.3 – 1.9. Only for the thick shunted device, the mobility is overestimated clearly. The cases with field-enhanced mobility are fitted with the Murgatroyd extension and result in underestimated mobility of 30 – 80%.
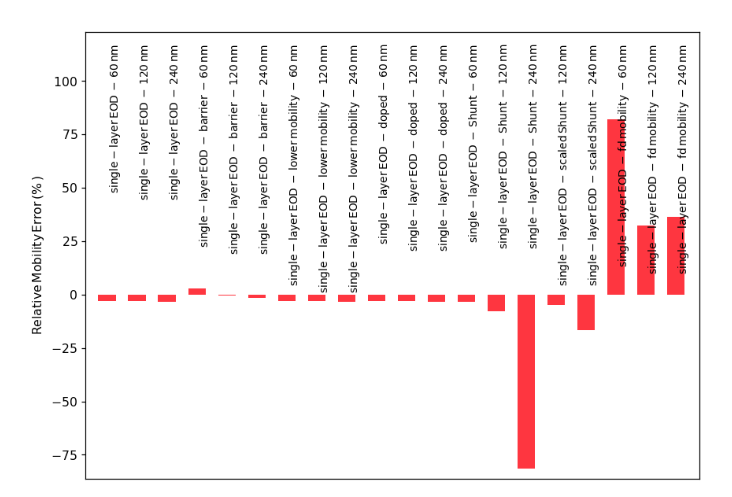
Fig. 6. Relative error of the extracted mobility using the MG equation.
Not shown in Figure 6 is the determined mobility from the reverse current in Figure 3b (injection barrier of 0.3 eV), which is 20 (240 nm thick device) – 500 times (60 nm thick device) lower than the input value! Such a strong thickness scaling of the extracted mobility would in-turn be a good indication that the value is affected by an energetic barrier. In contrast, the presence of an injection barrier is not always retrievable from the slope alone. The presence of trap states can increase the slope again, yielding to an overall value of 2 which is then mistakenly interpreted as perfect MG behaviour. [12]
Conclusions
We presented an overview of electrical characterisation techniques to determine the charge carrier mobility and outlined in more detail the analysis of the IV curve of a single-carrier device. The most common technique for organic semiconductors is to fit the Mott-Gurney model to the measured data. Therefore, we analyse this model's limitations by fitting the MG equation to simulated JV data. The method works well for simple device structures, but care must be taken when shunts, large injection barriers and/or doping are present.
Based on our analysis, we came up with the following take-home messages for experimentalists:
Plot dlogJ/dlogV vs. voltage: The absolute slope value can give a first indication.
If it is beyond 2, a field-enhanced mobility formula is advisable. Still, trap states can affect the model.
If the slope is closer to 1, this could indicate that the layer of interest is (unintentionally) doped. But it could also be an injection limitation. The trend with voltage eventually points towards an unintentionally doped layer.
Plot JL and JL^3 vs. voltage: Potentially identify high unintentional doping and injection barriers.
A slope <2 is often observed and does not necessarily mean that the MG law yields wrong results.
Extracted mobility values that show a clear thickness dependence indicate issues with the devices: injection barriers (> 0.2 eV), high doping densities (> 1e18 cm-3) and/or shunts.
References
[1] M. Z. Szymanski, B. Luszczynska, J.-M. Verilhac, P. Reiss, and D. Djurado, “Simplified transient space-charge-limited current measurements of mobility using transimpedance amplifier,” Org. Electron., vol. 14, no. 1, pp. 230–235, Jan. 2013
[2] J. Scott, S. Ramos, and G. Malliaras, “Transient space-charge-limited current measurements of mobility in a luminescent polymer,” J. Imaging Sci. Technol., vol. 43, no. 3, pp. 233–236, 1999,
[3] G. Juška, K. Genevičius, K. Arlauskas, R. Österbacka, and H. Stubb, “Charge transport at low electric fields in π-conjugated polymers,” Phys. Rev. B, vol. 65, no. 23, p. 233208, 2002.
[4] A. Pivrikas, N. S. Sariciftci, G. Juška, and R. Österbacka, “A review of charge transport and recombination in polymer/fullerene organic solar cells,” Prog. Photovoltaics Res. Appl., vol. 15, no. 8, pp. 677–696, 2007.
[5] D. J. Pinner, R. H. Friend, and N. Tessler, “Transient electroluminescence of polymer light emitting diodes using electrical pulses,” J. Appl. Phys., vol. 86, no. 9, pp. 5116–5130, 1999
[6] N. D. Nguyen, M. Schmeits, and H. P. Loebl, “Determination of charge-carrier transport in organic devices by admittance spectroscopy: Application to hole mobility in a-NPD,” Phys. Rev. B, vol. 75, no. 7, p. 075307, 2007,
[7] M. Schmeits, “Electron and hole mobility determination in organic layers by analysis of admittance spectroscopy,” J. Appl. Phys., vol. 101, no. 8, p. 084508, 2007
[8] A. Melianas and M. Kemerink, “Photogenerated Charge Transport in Organic Electronic Materials: Experiments Confirmed by Simulations,” Adv. Mater., vol. 31, no. 22, p. 1806004, 2019
[9] P. N. Murgatroyd, “Theory of space-charge-limited current enhanced by Frenkel effect,” J. Phys. D. Appl. Phys., vol. 3, no. 2, p. 308, 1970,
[10] G. A. H. Wetzelaer, “Improved Determination of the Mobility and Built-In Voltage in Asymmetric Single-Carrier Devices,” Phys. Rev. Appl., vol. 13, no. 3, p. 034069, 2020.
[11] J. C. Blakesley et al., “Towards reliable charge-mobility benchmark measurements for organic semiconductors,” Org. Electron., vol. 15, no. 6, pp. 1263–1272, 2014.
[12] J. A. Röhr, D. Moia, S. A. Haque, T. Kirchartz, and J. Nelson, “Exploring the validity and limitations of the Mott–Gurney law for charge-carrier mobility determination of semiconducting thin-films,” J. Phys. Condens. Matter, vol. 30, no. 10, p. 105901, Mar. 2018.
[13] A. A. Grinberg and S. Luryi, “Space-charge-limited current and capacitance in double-junction diodes,” J. Appl. Phys., vol. 61, no. 3, pp. 1181–1189, 1987.
[14] J. A. Röhr, T. Kirchartz, and J. Nelson, “On the correct interpretation of the low voltage regime in intrinsic single-carrier devices,” J. Phys. Condens. Matter, vol. 29, no. 20, p. 205901, 2017
[15] G. A. H. Wetzelaer and P. W. M. Blom, “Ohmic current in organic metal-insulator-metal diodes revisited,” Phys. Rev. B, vol. 89, no. 24, p. 241201, 2014
[16] N. F. Mott and R. W. Gurney, Electronic Processes in Ionic Crystals. Oxford: Oxford University Press, 1940.
[17] G. Zuo, Z. Li, O. Andersson, H. Abdalla, E. Wang, and M. Kemerink, “Molecular Doping and Trap Filling in Organic Semiconductor Host-Guest Systems,” J. Phys. Chem. C, vol. 121, no. 14, pp. 7767–7775, 2017.
[18] N. Felekidis, A. Melianas, and M. Kemerink, “Automated open-source software for charge transport analysis in single-carrier organic semiconductor diodes,” Org. Electron., vol. 61, no. March, pp. 318–328, 2018.
[19] N. B. Kotadiya, A. Mondal, S. Xiong, P. W. M. Blom, D. Andrienko, and G.-J. A. H. Wetzelaer, “Rigorous Characterization and Predictive Modeling of Hole Transport in Amorphous Organic Semiconductors,” Adv. Electron. Mater., vol. 1800366, p. 1800366, 2018.
[20] G. Zuo, M. Linares, T. Upreti, and M. Kemerink, “General rule for the energy of water-induced traps in organic semiconductors,” Nat. Mater., vol. 18, no. 6, pp. 588–593, 2019.